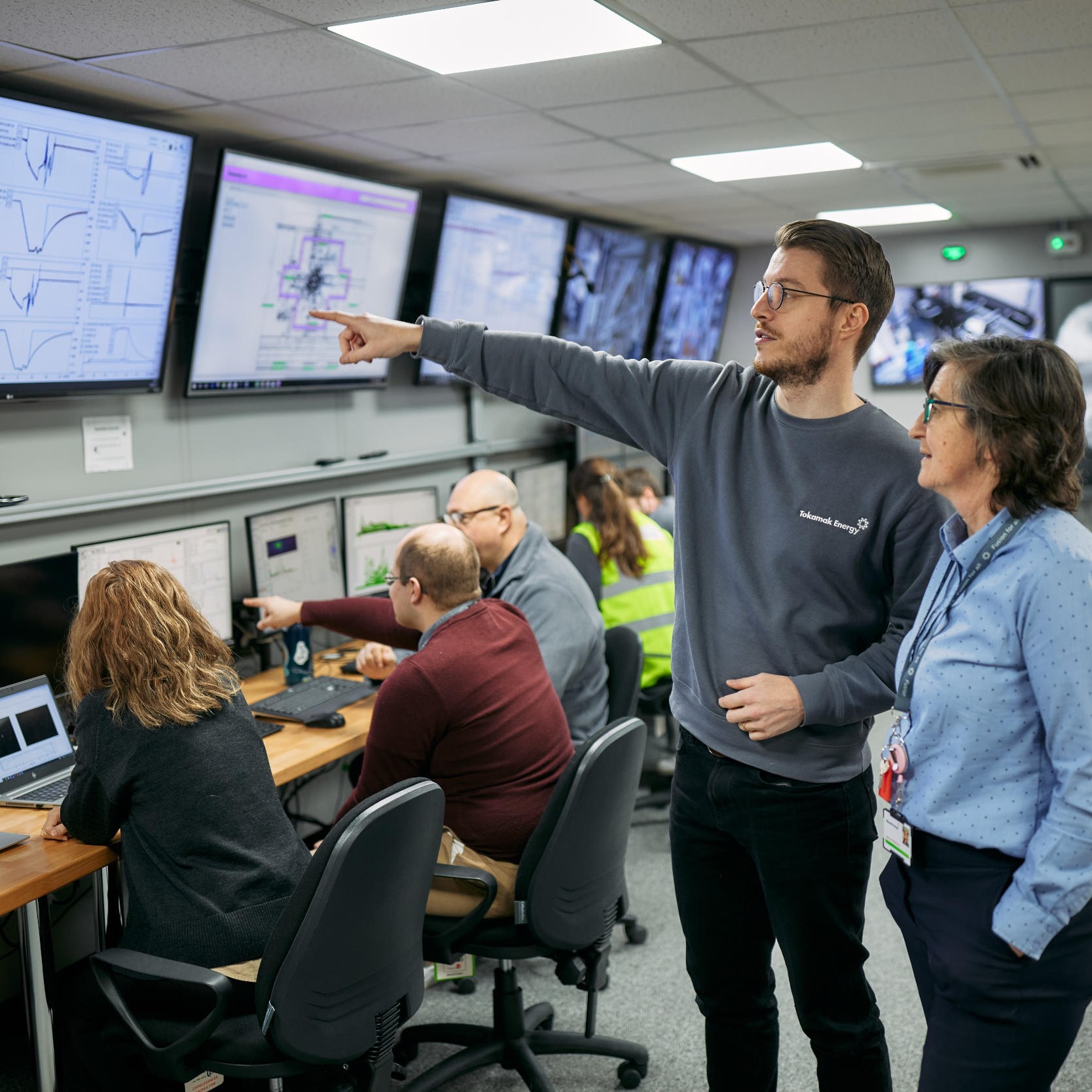
[ad_1]
//php echo do_shortcode(‘[responsivevoice_button voice=”US English Male” buttontext=”Listen to Post”]’) ?>
Tokamak Energy’s ST40 compact high-field spherical tokamak has achieved ion temperatures greater than 100 million degrees Kelvin (8.6 keV), a crucial milestone in fusion technology. A peer-reviewed scientific paper on this achievement has recently been published by the Institute of Physics (IOP).
Ion temperatures more than 5 keV have not been previously attained in any spherical tokamak and have only been produced in considerably bigger devices with far higher plasma heating power, according to the paper. The corresponding fusion triple product is calculated to be about 6×1018 m-3keVs. This result reveals for the first time that ion temperatures suitable for commercial magnetic confinement fusion may be reached in a small high-field spherical tokamak, and bodes well for high-field spherical tokamak-based fusion power facilities.
Many advantageous characteristics of spherical tokamaks make them an appealing choice for commercial fusion power production. Spherical tokamaks have a plasma aspect ratio (the ratio of the main to minor radii of the plasma torus) of around two or less, and demonstrate improved stability and enhanced confinement qualities compared to tokamaks with a large plasma aspect ratio.
Recent breakthroughs in the production of high-field magnets constructed from a high-temperature superconductor (HTS), along with the fundamentally advantageous features of the spherical tokamak, pave the way for smaller fusion power plants that may be less expensive. Oxford-based Tokamak Energy is building commercial fusion power plants using this methodology, developing spherical tokamaks with HTS magnets to commercialize fusion worldwide. The U.K. Atomic Energy Authority spun out the firm in 2009 and now employs over 240 U.K. and international professionals.
In an exclusive interview with EE Times, Steven McNamara, science director at Tokamak Energy, said that the company has 10 years’ experience in designing, building, operating and validating record-breaking results using tokamaks. However, there are still technologies they need to invent and challenges to overcome on their roadmap, McNamara noted.
“We have broken these down into 11 building blocks, including fuel cycle, high-temperature superconducting magnet technology, plasma facing components and shielding,” he said. “We believe on-grid power in the 2030s is viable. The U.S. Department of Energy, a major player, has set the target of a fusion power plant on grid within 10 years. The U.K. is backing fusion. Once you get big countries behind it, things like SpaceX happen. Fusion will happen.”
ST40 and the fusion process
The fusion process powers the sun and stars, emits no carbon, and has a virtually limitless fuel supply while still being secure and safe. When deuterium and tritium–two different types of hydrogen, are heated to create a controlled plasma at high temperatures (hotter than the sun’s core), they combine to make helium and release energy that may be used to generate heat and power.
Strong magnets are used to contain this heated plasma inside a device known as a “tokamak,” which is ring-shaped. Fusion energy may be utilized to produce heat and electricity in the same way as current power plants. Per kilogram of fuel, fusion is a million times more energy-efficient than burning coal, oil or gas.
“One kilogram of fusion fuel releases the same amount of energy as burning about 10 million kilograms of coal,” McNamara said. “Nothing can compete with it. Fusion will support the fight against climate change and complement, not compete with, other renewables as part of a sustainable net zero future.”
One-hundred million degrees Celsius is important for fusion energy because it’s above the threshold required for fusion energy. “The fusion fuels we intend to use in power plants—deuterium and tritium—fuse together at this temperature, releasing the huge amounts of energy that could be turned into electricity for the grid,” he added.
The ST40 tokamak is hotter than the sun’s center by a factor of six. A range of diagnostic systems are used to measure different parameters, which are then used to control, optimize and understand the plasma.
For example, real-time magnetic control and post-pulse magnetic reconstruction utilize all magnetic field and flux sensors. Their in-house reconstruction code determines the plasma centroid position and form in real time.
Two interferometers—a sub millimetre (SMM) system at 1 mm and a near-infrared (NIR) system at 1 μm + 1.5 μm—measure electron density along radial and tangential midplane lines of sight. A visual survey spectrometer and line-filtered diodes monitor low-Z impurities and Bremsstrahlung, while a tangential viewing soft x-ray (SXR) camera with a 10 μm Be-window measures radiated power.
A 15-channel neutral particle analyzer measures neutral hydrogenic particle fluxes at 1–41 keV to determine the rapid ion distribution function. High-resolution fast visible (10 kHz) and Dα (1 kHz) cameras view the main chamber and upper/lower divertors. Infrared cameras capture the center column and divertor zones.
A high-spectral resolution x-ray crystal spectrometer (XRCS) diagnostic analyzes electron and ion temperatures in the core area over a single LOS gazing radially across the midplane. Each shot needs a short prefill puff of argon.


“One method uses a technique called spectroscopy, where light emitted from the plasma is used to determine its temperature,” McNamara said. “ST40 has since been upgraded with a new Thomson scattering high-powered laser system, which gives even greater insights into plasma behaviour, by measuring both temperature and density at many points. This is important for informing and perfecting power plant designs.”
The ST40 is a small, copper-magnetized high-field spherical tokamak. ST40’s plasmas have major radii of 0.4–0.5 m, aspect ratios of 1.6–1.9, plasma currents of 0.4–0.8 MA, and on-axis toroidal magnetic fields of 1.5–2.2 T. Two neutral beams injected tangentially in the co-current direction heat plasma at 0.9 MW at 55 kV, and 0.7 MW at 24 kV when operated in deuterium.
Carbon ion temperatures of 9.2 keV were determined using a charge exchange recombination spectroscopy (CXRS) system, with the most central line of sight slightly off the magnetic axis by injecting 1.6 MW of deuterium neutral beams into a deuterium plasma of volume 0.9 m3, plasma current of 0.6 MA, and on-axis toroidal magnetic field of 1.9 T, according to the paper.
This equates to a primary ion temperature of 9.6 keV calculated using TRANSP and a central impurity temperature of 9.8 keV determined using forward diagnostic models. The uncertainty of the inferred values for the central temperatures (impurity and hydrogenic) is below 10%, but the uncertainty of the CXRS measured value is below 5%. This is the greatest ion temperature ever produced in a spherical tokamak, or any tokamak of comparable size, according to researchers.
However, only devices with plasma volumes more than 15× bigger than ST40 and with significantly higher plasma heating power were able to achieve equivalent ion temperatures in the past, according to the scientific team. These temperatures were attained in hot-ion mode settings, where the ion temperature is often two or more times higher than the electron temperature.
McNamara explained that the process of injecting high-energy beams of neutral hydrogen is used to get the plasma to an extremely hot temperature—it interacts with the plasma and transfers that energy. “Another way to do it is to use electromagnetic waves tuned to frequencies that resonate with the particles in the plasma to transfer energy,” he added.

McNamara believes compact spherical tokamaks—cored apple rather than ring doughnut shape—with HTS magnets is the optimal route.
“There are many different approaches. The spherical tokamak is much more efficient than the traditional design, with lower capital investment, operating costs and smaller footprint,” he said. “We have recently announced our future roadmap for the next phase of development towards competitive, commercial fusion and our ST-E1 fusion pilot plant will demonstrate the capability to deliver electricity into the grid in the early 2030s—up to 200 MW of net electrical power. We’re not about just proving we can do fusion—that isn’t good enough. Our mission is to have a solution that can be low cost, and genuinely deployable in many countries, because the world needs fusion.”
Potential applications
Fusion power plants can be connected to a traditional turbine to produce electricity, but as McNamara explained, there are multiple industrial uses beyond grid.
“Any process needs heat, like melting metal, water desalination, hydrogen, or sustainable aviation fuel production,” he said. “It could be a huge growth market that can be enabled if you have this deployable fusion power and heat source. Renewables are vital, but you also need dependable, reliable power you can turn on when you want. When the sun isn’t shining, or wind isn’t blowing.”
The ST40 is the smallest tokamak to reach this 100 million degree temperature, demonstrating the spherical tokamak’s potential as an efficient, practical and cost-effective option for commercial fusion power plants, according to scientists.
[ad_2]